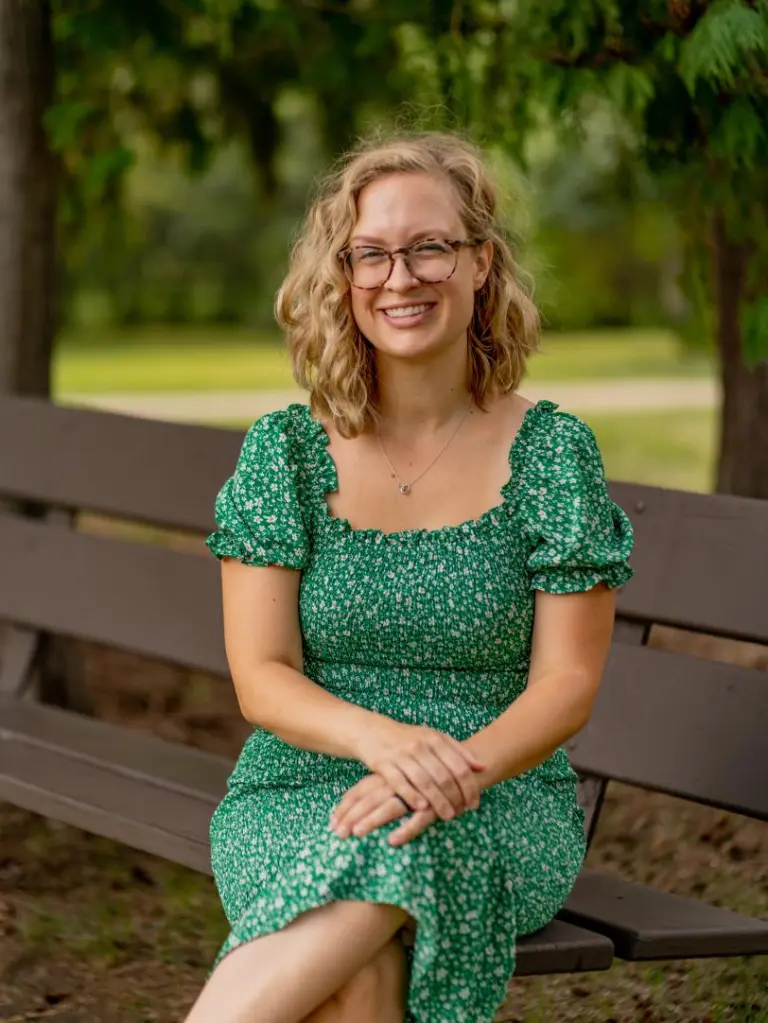
Ashley James (Ashlyn George)
Ashley James is fascinated by her toxicology research because it combines biology, chemistry, physics and morbid topics like poisonings that affect the environment and world health. She also loves the unexpected twists. “We’ve been surprised so many times by what we’ve found. It’s been a fun, wild ride,” she said.
As a PhD student and now postdoctoral research fellow from the University of Saskatchewan, James’ research on mercury poisoning in animals and humans uses X-rays produced by the Stanford Synchrotron Radiation Lightsource (SSRL) at the Department of Energy’s SLAC National Accelerator Laboratory.
For her work, James will receive the 2023 Melvin P. Klein Scientific Development Award during the 2023 SSRL/LCLS Annual Users’ Meeting held at SLAC September 24-29.
“The SSRL-based research of Dr. James addresses a global health question with breakthrough discoveries while demonstrating state-of-the-art methodology for her discipline, thus bearing all the hallmarks of ‘outstanding research accomplishments by a new investigator’ that the award is intended to recognize,” wrote her PhD supervisors Graham George and Ingrid Pickering, Canada Research Chairs and professors at the University of Saskatchewan, in a nomination letter for the award.
James said she felt surprised, excited and honored by SSRL when she found out about winning the award. “It’s awesome to be included in the list of Klein awardees, alongside the diverse projects and incredible scientists who’ve won in the past,” she said.
Re-thinking the Minamata mass poisoning
As a PhD student, James studied the mass poisoning of thousands of people who ingested mercury by eating tainted fish and shellfish from the Minamata Bay in Japan during the late 1950s and 1960s. This famous, deadly tragedy was caused by a chemical plant dumping mercury-contaminated industrial waste into the bay – as demonstrated by a physician working for the factory who fed cats food laced with the industrial waste to confirm that it was responsible for the neurological disease.
Because the chemical plant used inorganic mercury in its processes, scientists initially believed that the contaminated waste contained inorganic mercury. The idea was that this inorganic mercury was transformed in the environment into a common and more toxic form of organic mercury called methylmercury.
James and her collaborators investigated the Minamata poisoning by studying preserved samples from a cat in the historic study. First, they showed that the mercury in the cat’s brain tissue was mostly organic by performing studies at SSRL’s X-ray spectroscopy (XAS) beamline 7-3 and high-energy-resolution fluorescence detection (HERFD-XAS) beamline 6-2, with help from SLAC scientists Matthew Latimer, Thomas Kroll and Dimosthenis Sokaras.
“These synchrotron techniques allowed us to look at historical samples with X-ray eyes to determine what mercury compounds existed in the cat’s brain tissue, which then told us a lot about its toxicology,” explained James. “HERFD-XAS has been particularly useful because its higher resolution enhances the shape of the mercury spectra, so we can fit the complex mixture of compounds with more confidence.”
The team then used computer-based calculations to model the chemical plant’s processes. Instead of methylmercury, their computational chemistry studies predicted that the factory released a different organic mercury compound called alpha-mercuri-acetaldehyde, whose toxicology has not been studied. Their findings challenged the long-standing view of what form of mercury poisoned the human population in Minamata.
The ensuing controversy attracted media attention and some scientific criticism, which was a bit overwhelming and stressful for James as an early-career PhD student. However, she handled it like a veteran, according to her nomination letters. Her supporters described the criticism published in letters to the journal as personal and unscientific. And they praised James’ response as excellent and surgically precise.
“Science is meant to create debate and it certainly did that,” said James. “But our main point was that it is important to study the toxicology of organic mercury compounds other than methylmercury, because they may have important environmental and health impacts.”
Comparing acute and chronic mercury poisoning in humans
Her second PhD research project investigated the more prevalent issue of chronic mercury exposure due to a lifetime of eating marine fish with low levels of methylmercury, which can lead to as much as ten times higher concentrations of mercury in the brain. Specifically, she used the same X-ray spectroscopy techniques and SSRL facilities as the Minamata project to study brain samples from residents of the Seychelles islands.
She compared these results to similar beamline studies on two historic acute organic mercury poisoning cases, which involved a short-term exposure of large concentrations of organic mercury. All brain tissue samples for her PhD work were acquired through her collaborators at the University of Rochester.
“We wanted to see if there was a difference in the chemical form of mercury found in chronic versus acute human exposure cases,” said James. “Honestly, we found the complete opposite of what we expected.”
The human body uses a chemical process called demethylation as a defense mechanism to slowly turn organic mercury into less toxic inorganic mercury. The researchers therefore thought people showing no evident symptoms of mercury poisoning would have mostly inorganic mercury that had been demethylated, James explained. Instead, they found individuals with chronic exposure had low concentrations of mercury in their brains, but it was entirely organic.
Similarly, the scientists predicted individuals after acute poisoning would have less time to demethylate the mercury, meaning they would have high levels of organic and low levels of inorganic mercury. Instead, they found complex mixtures with low concentrations of organic and high concentrations of inorganic mercury.
“The takeaway of this crazy twist is that it could be misleading to use acute exposure studies to understand the vast majority of human exposures that are chronic in nature,” said James. “Over a billion people worldwide depend on fish as their primary or sole source of protein. So, better understanding the ramifications of ingesting fish that may contain low levels of mercury is an important global food security question.”
As a postdoc, James is extending her mercury toxicology research. She is now studying the role of metals in multiple sclerosis using a diverse range of SSRL X-ray spectroscopy and X-ray imaging beamlines.
“Dr. James’ remarkably impactful research is further distinguished by her use of an incredible range of different techniques — from advanced X-ray spectroscopy methods at SSRL to quantum chemistry, alongside more conventional toxicology methodology,” said George and Pickering. “This work represents one of the very first demonstrations of these techniques to her field.”
The Klein award is named in honor of the late Melvin P. Klein, a world-renowned biophysicist at Lawrence Berkeley National Laboratory and the University of California, Berkeley and a longtime user at SSRL.
For questions or comments, contact the SLAC Office of Communications at communications@slac.stanford.edu.
SSRL is an Office of Science user facility.
This is a a reposting of my news feature courtesy of SLAC National Accelerator Laboratory.